In silico: advancing battery chemistry through supercomputer simulation
19 August, 2021
With Northvolt’s access to the Vega supercomputer, demonstration of a powerful new approach to battery research & development arrives. In search of new chemistries and formulations, the work ahead holds promise for higher performance batteries to drive forward the electric revolution.

Located in Maribor, Slovenia, Vega is one of the most powerful computers in Europe. The petascale supercomputer is the first to come online within the European High Performance Computing Joint Undertaking, and the allocation of 2.5 million processing hours of the Vega supercomputer to Northvolt represents the first time that access to a EuroHPC system has been granted to private industry.
Beginning this autumn, Northvolt’s Vega processing time will be committed towards the advancement of lithium-ion electrochemistry to deliver higher performance battery solutions to accelerate the transition to a decarbonized society.
Supporting Northvolt’s application for access to Vega was the EuroCC National Competence Centre Sweden (ENCCS), whose Director, Dr. Lilit Axner, tells Northvolt: “This is the first industrial use of the Vega system, and it’s a perfect use case – to apply this technology to explore a field of chemistry in a cleaner, smarter way and advance new solutions for a sustainable energy future.”
“The project will yield direct results, but it’s also a valuable proof of concept, one by which we intend to demonstrate the simulation approach within the field of battery electrochemistry research. In this project, the focus is on electrolyte, but this is just one component within the battery cell, and we can imagine all others would benefit from future research in simulation.”
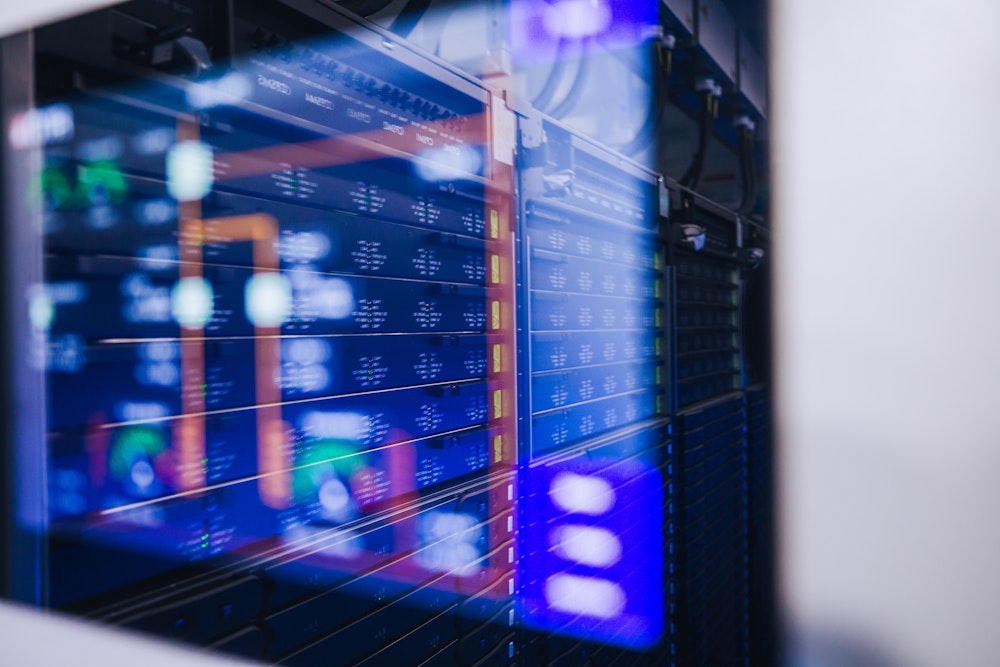
The digital experiment
The foundation to science has of course been the experiment – controlled studies conducted either in the field or laboratory. With the advance of exceedingly powerful computers, however, an increasingly attractive option is to experiment in simulation – to model molecules, materials or systems and their behaviors. This field of computational chemistry, grounded in research undertaken not in labs but rather in silicon computer chips – in silico as it were – brings great opportunities for R&D.
While in silico research isn’t about to replace laboratory experimentation, the benefits of its use as a complement to traditional approaches are clear. Most battery cell research today is laboratory-based, and it’s undertaken at several levels. At the highest level, we can consider the whole cell – exploring how physical design or manufacturing decisions can be optimized to improve cell performance for instance. Another branch of research is more fundamental – concerned with how the chemistry of key materials within the cell can be designed and controlled at the molecular level. It’s this kind of fundamental research that is particularly well-suited to explore through in silico simulation.
With fundamental research, we often focus on molecular dynamics relevant to the design and functioning of active materials of electrodes – the nickel-manganese-cobalt based material of cathode, or graphite composites of anode. Or alternately, as is the focus in Northvolt’s project to be undertaken at VEGA, the design and formulation of electrolyte – the substance which fills a battery cell and enables the movement of ions through the cell, but which can be too easily overlooked as a key performance determining feature of the battery cell.
“The insight that simulation offers is much greater than what thousands of experiments can provide. And the more computing power you have, the more simulations you can design and carry out.”
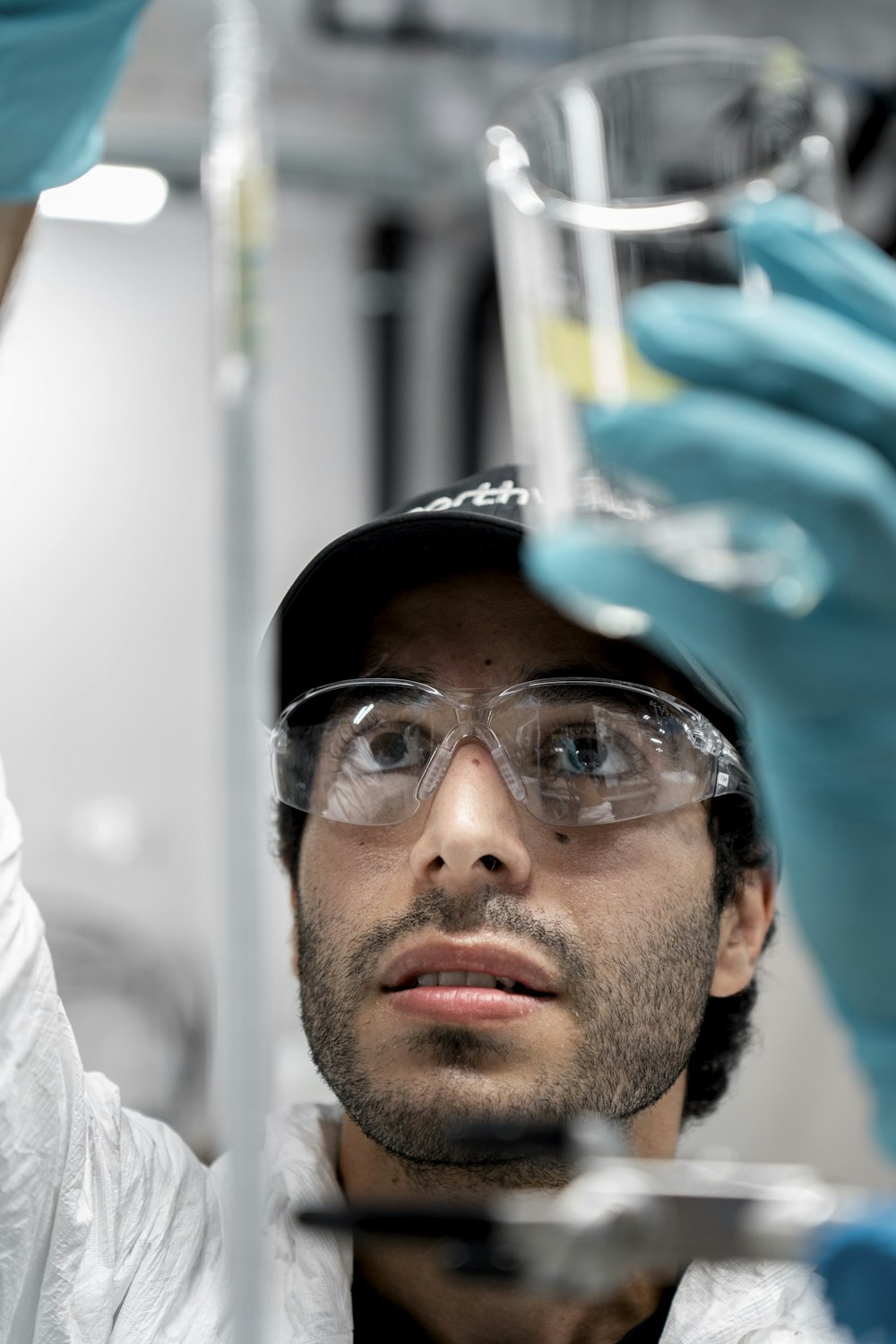
Laboratory research exploring cathode materials at Northvolt Labs.
At the level of fundamental research, we’re designing for chemical reactions, and we’re often looking at the behaviour of single molecules, or perhaps a set of molecules, to see about optimizing a reaction in some way or other with the aim of improving cell performance, lifespan or safety.
A route to optimizing reactions could be to adjust molecular characteristics of a material. And this can of course be done in a laboratory, as Roberto Di Remigio, PhD of theoretical and computational chemistry at ENCCS explains: “one can run a physical experiment to see how changes to the molecule impact the reaction. And we can run multiple experiments – adjusting some parameter of the system each time to determine the effect of this change on the experimental results. Those parameters could be temperature, the composition of sample material, its salt concentration or pH, the addition of some new molecule and so forth.”
The approach has brought us a long way. We need only look at the evolution of lithium-ion batteries over recent decades and the state-of-the-art for evidence of this. But it’s not without constraints.
“There are certainly limitations to this approach,” says Roberto. “To begin with, it’s a costly, complex and lengthy process – especially if you’re looking to run multiple experiments. Keep in mind too that from both qualitative and quantitative perspectives, the level of insight gained through laboratory experiment is limited by your access to the instruments necessary to reveal details in the chemistry.”
The same experiment undertaken in simulation, however, unfolds quite differently. From a computational point of view, changing those parameters is simply a matter of writing a different input file and running the simulation again.
Lilit captures the advantages neatly, saying: “experimenting in silico, you don't need to fight with the realities of lab testing. The synthesis procedure required to build a new material or formulation can be incredibly complex, requiring hundreds of steps. That also means hundreds of reactions, with each reaction perhaps producing unwanted byproducts, or chemical waste that is expensive to process. All these steps are removed in a simulation, which has a massive impact on investment of cost and time.”
Further benefits of the simulation approach emerge when we consider how working in silico reduces the human resources required for experimentation. The training for organic chemistry is incredibly hard and the expertise is limited, Lilit explained – adding further limitations to a group’s ability to conduct fundamental research. “Relatedly, a starting point for complex experiments oftentimes includes production of complex molecules which themselves even fewer people yet can produce, and which take time to produce from smaller building blocks.”
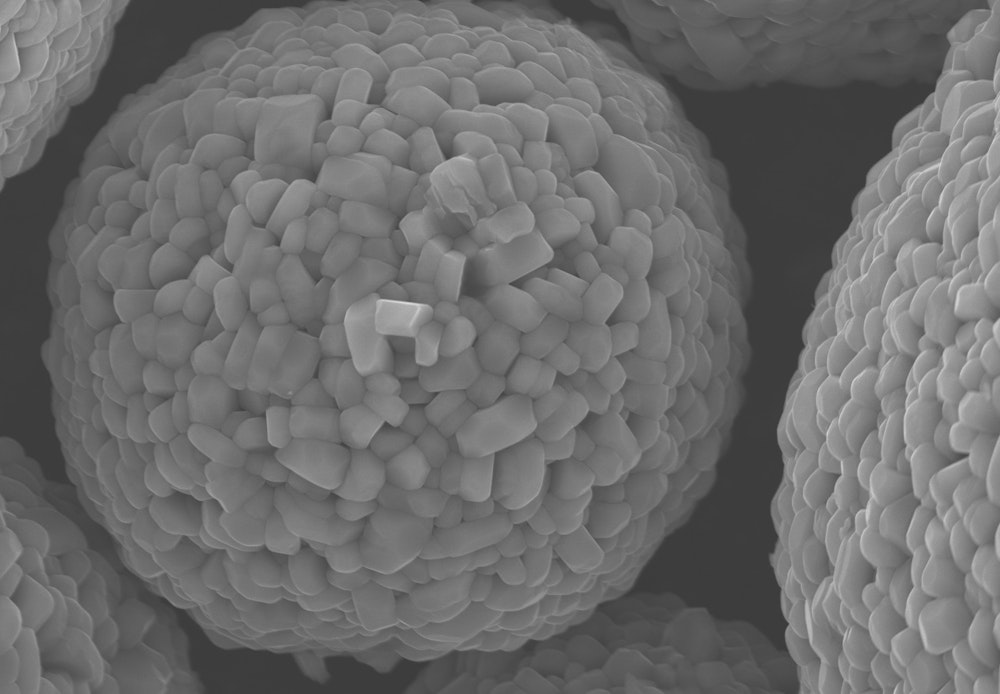
Imaging active cathode material with a scanning electron microscope.
The need for power
Reductions in expense, human resources and time are all immensely valuable. But a major strength of running experiments in silico arrives with the ability of computers to run multiple experiments in parallel. The analysis of results also benefits – more calculations and more precise calculations can be made.
But there is a catch: “from a processing perspective, running these experiments and analysis of output data demands an extraordinarily large amount of computer processing power,” says Roberto. “And at the level of atomistic simulation, there are aspects one might not consider – the larger the molecule that you want to simulate, for instance, the more computing power you need.”
It’s here where the supercomputer comes in.
“Altogether, it’s only possible for us to run these studies with some incredible powerful computers, the likes of which Vega provides.”
Petascale computers, like Vega, are some 1,000 times faster than even their predecessor terascale supercomputers and can perform over 1,000 trillion operations per second. As for Vega itself, its 6.9 petaflops enables a staggering 6.9 million billion calculations per second.
Ultimately, the ambition is that the entire in silico experiment process flow – spanning simulation through to analysis – is automated. It is precisely this, both development and demonstration of an automated simulation workflow, which Northvolt will explore through its Vega project.
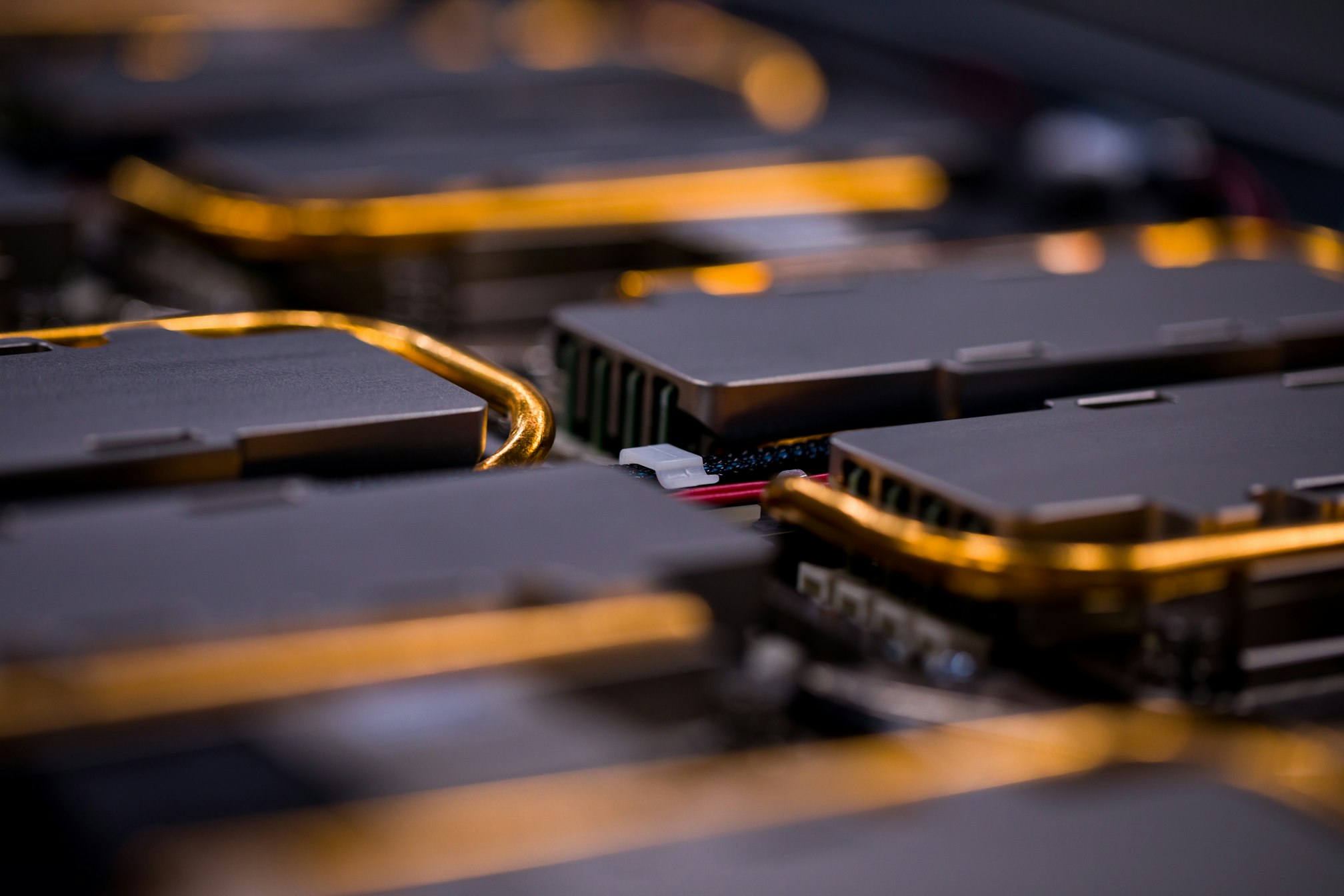
At 6.9 petaflops, the vega supercomputer can run 6.9 million billion calculations per second.
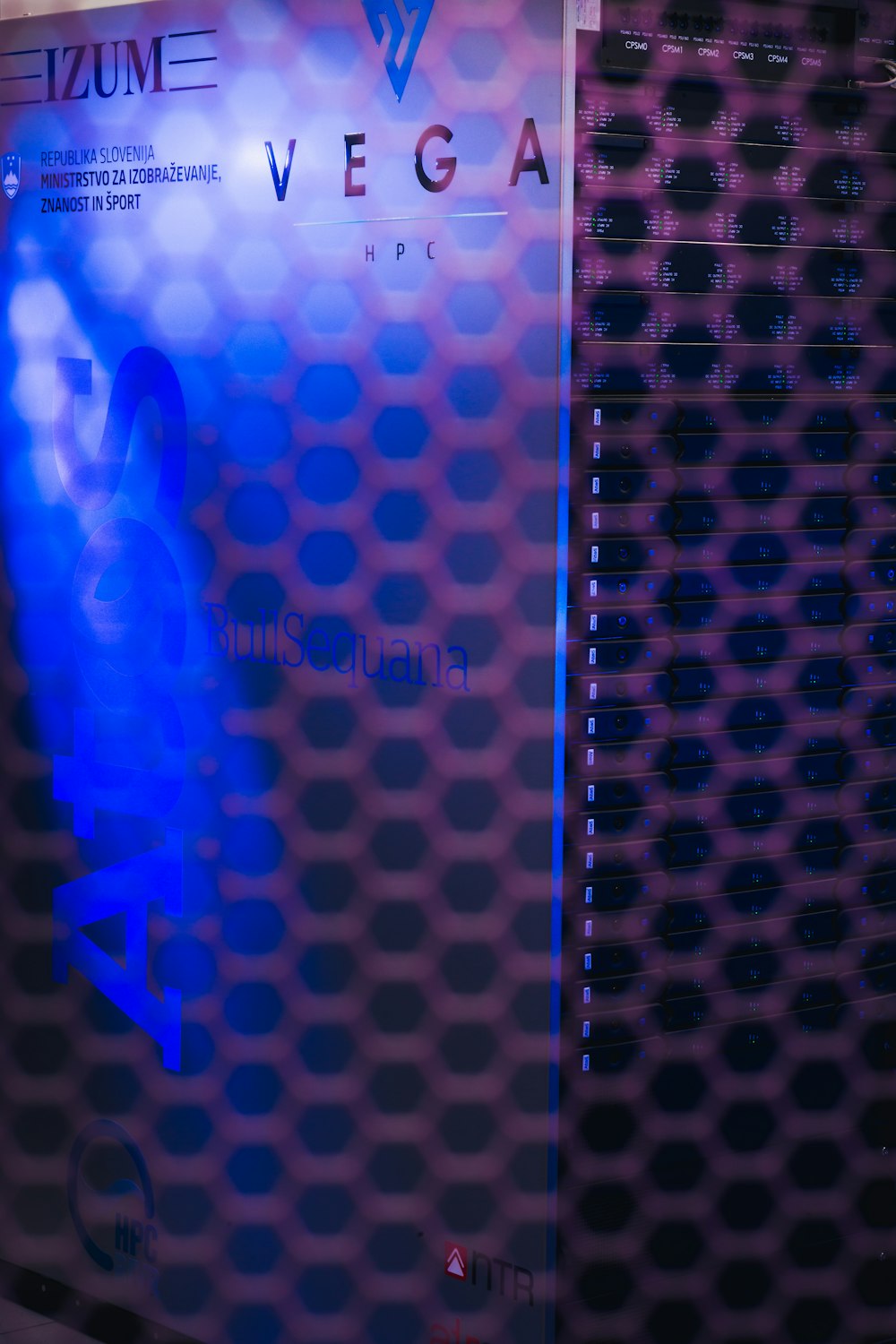
Bending the rules of chemistry
A final aspect to supercomputer-based experiment is perhaps the most intriguing, and it’s something that Lilit is particularly excited for.
“The simulated environment of in silico experiments allows for more daring research. What I mean by that is that you're more flexible to play around with chemical compositions, particularly with compounds are extremely hard to make, or even hypothetical compounds which might not exist in real life.”
In this sense, in silico simulations re-write the rules governing what can and cannot be investigated. Playing around with hypothetical molecules or formulations might seem strange, but it’s an approach which can reveal data points and insights that hint at a previously unknown or never-before considered directions for research.
“For example, in simulation, we can see what happens if we add a new functional grouping molecule – a fragment of a molecule, of 3 to 5 atoms, with characteristic functionality – or introduce a positive charge on this atom in the molecule… slight adjustments which would costly, time-consuming or outright impossible in real-life, but can be quite easily explored in simulation,” says Lilit.
And even if it is not the objective to develop a new molecule, simulations provide a platform to accelerate the search and identification of new candidate materials for formulations, or to screen large sets of molecules based on some property of interest.
For Northvolt, the simulated playground of in silico experimental testing opens a world of opportunities. In the most immediate timeframe, Northvolt will be committing its processing hours on the Vega supercomputer to the optimization of a new electrolyte formulation. And we need only look towards the work of Northvolt’s Cuberg to see the great implications that a novel electrolyte can have in terms of enabling higher performance cells.
While for the time being Northvolt is exploring in silico experimentation of single components of the battery, in the not-too-distant future the plan is to increase the complexity of the systems under investigation. Already in 2022, Northvolt will expand its focus into electrode interfaces, and in a few more years aims to characterize and simulate a complete nanobattery – featuring anode, cathode and electrolyte – entirely in silico.
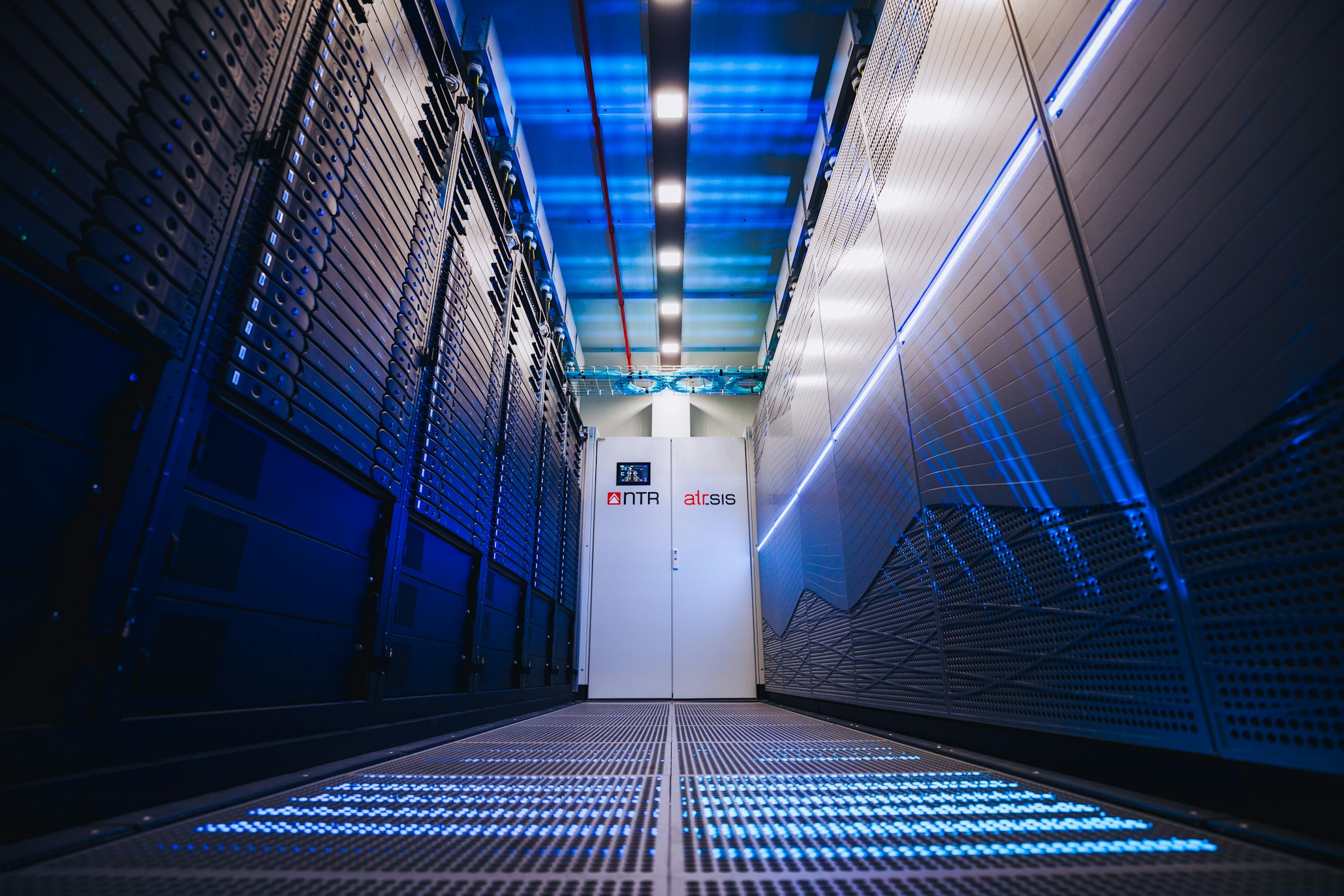